🌱 Plant Information from the Field of Master Mind Quantum Mechanics (MMQM) 🔬
Shared from the research of: Joseph Mercado 🙇
Article Author: Dr. Johanna Deinert, MD 👩
Content Contributor: Royal Society Publishing 🌎
Blog Post #1163 📌
Re: Origins of Quantum Biology 🌀
Date and Time: Thursday, April 1, 2021 at 3:05 p.m. ⏰
Dear Quantum Lover,
Abstract:
Quantum biology is usually considered to be a new discipline, arising from recent research that suggests that biological phenomena such as photosynthesis, enzyme catalysis, avian navigation or olfaction may not only operate within the bounds of classical physics but also make use of a number of the non-trivial features of quantum mechanics, such as coherence, tunnelling and, perhaps, entanglement.
However, although the most significant findings have emerged in the past two decades, the roots of quantum biology go much deeper—to the quantum pioneers of the early twentieth century.
We will argue that some of the insights provided by these pioneering physicists remain relevant to our understanding of quantum biology today.
1. Introduction:
There is growing evidence that a number of specific mechanisms within living cells make use of the non-trivial features of quantum mechanics, such as long-lived quantum coherence, superposition, quantum tunnelling and even quantum entanglement—phenomena that were previously thought to be relevant mostly at the level of isolated molecular, atomic and subatomic systems, or at temperatures near absolute zero, and were thereby not thought to be relevant to the mechanisms responsible for life.
It is important at the outset of this review, and before we delve into the origins of quantum biology, to make clear that what is currently understood by the term ‘quantum’ in quantum biology does not simply mean quantization: the discretization of electron energies to account for chemical stability, reactivity, bonding and structure within living cells.
Clearly, quantization applies to all matter at the microscopic scale and has long been assimilated into standard molecular biology and biochemistry.
Today, quantum biology refers to a small, but growing, number of rather more specific phenomena, well known in physics and chemistry, but until recently thought not to play any meaningful role within the complex environment of living cells.
For an up-to-date discussion on recent advances in quantum biology and what it means, see for example.
One of the most celebrated examples of the non-trivial role that quantum mechanics might be playing in biology is the claimed long-lived quantum coherence observed in the transport of exciton energy in photosynthesis.
While this subject remains controversial, a more established role for quantum mechanics is found in the tunnelling of electrons and protons in enzyme catalysis.
Beyond these examples of quantum biology, quantum entanglement has been implicated in avian navigation, while quantum tunnelling has been proposed to be involved in olfaction and mutation.
More speculatively, some have suggested a link between quantum coherence and consciousness, though this view has little support within the neurobiology community.
But while the current interest in quantum biology and the study of the phenomena and mechanisms mentioned above only emerged in the past two decades, the origins of the subject go much further back to the quantum pioneers of the early twentieth century. We will argue that some of the insights provided by a number of these physicists remain relevant to our understanding of quantum biology today.
Quantum biology’s origins are often traced back to 1944 and the publication of Erwin Schrödinger’s famous book, What is Life?
But even before this, several other quantum physicists had already made inroads into biology.
For example, the German physicist Pascual Jordan published a book a year before Schrödinger’s, entitled Physics and the Secret of Organic Life, in which he had posed the question ‘Sind die Gesetze der Atomphysik und Quantenphysik für die Lebensvorgänge von wesentlicher Bedeutung?’ (‘Are the laws of atomic and quantum physics of essential importance for life?’).
In fact, Jordan had been thinking about this question for over a decade and had been using the term Quantenbiologie since the late 1930s.
The murky origins that motivated and sustained his interest in quantum biology are inextricably linked to his political sympathies with Nazi Germany and play an important role in explaining why the field did not flourish further after the war ended.
Quantum biology was in fact born shortly after the development of quantum mechanics itself.
By 1927, the mathematical framework of the new quantum mechanics was in place, owing to the efforts of Bohr, Heisenberg, Pauli, Schrödinger, Dirac, Born, Jordan, Fermi and others.
Flushed with their success at taming the atomic world, and with the arrogance of youth on their side, many quantum pioneers strode out of their physics laboratories and away from their blackboards to seek new areas of science to conquer. Microbiology along with the emerging field of genetics and the chromosome theory of inheritance were still unexplored territories, and a growing number of biophysicists and biochemists began to show more than a passing interest in these subjects.
It was only natural therefore for many to ask whether the new atomic physics might also have something to say about the building blocks of life.
Advances in experimental physics around this time were also posing new questions.
Just as Robert Hooke’s microscope had opened up a new world of the very small in the mid-seventeenth century, so new techniques and key experiments in the decades between the two world wars were helping lay the foundations of an even smaller, molecular, biology. These included the discovery of X-ray mutagenesis by H.J. Muller in 1927, Theodor Svedberg’s measurement of the atomic weights of proteins by using his famous ultracentrifuge in the mid-1920s and, later, the crystallization of a virus by W.M. Stanley in 1935. These and other breakthroughs promoted a feeling of optimism that, with the tools of quantum mechanics, the secrets of life could finally be laid bare.
However, not everyone was so confident that the principles of physics and chemistry would be sufficient to explain biology. One such critic was Niels Bohr himself; and yet, as we shall see, it was Bohr’s pessimism regarding the importance of quantum mechanics in unlocking the secrets of life that would, paradoxically, influence and inspire the men who would lay the foundations of quantum biology.
At the same time as the quantum revolution was taking place in physics, enormous strides were being made in biology through neo-Darwinian synthesis, which brought together the rediscovered principles of Mendelian heredity with the mutations identified by Hugo de Vries and Thomas Hunt Morgan.
However, many mysteries remained, particularly surrounding the nature of the heritable material. Microscopic studies at the end of the nineteenth century had associated visible chromosome fibres with Mendel’s heritable factors, by this time called ‘genes’.
Biochemical studies had established that chromosomes consisted of proteins and nucleic acids; but how the genetic information was written into ordinary chemicals and then inherited remained a complete mystery.
Moreover, although the idea of vitalism, which maintained that there is some vital ‘life force’ that endows organisms with a special quality absent in inanimate matter, had retreated under the influence of nineteenth century advances, such as Wöhler’s synthesis of urea, many scientists and philosophers clung to the belief that some aspects of life required principles outside of classical science.
For example, in 1907, the French philosopher Henri Bergson first published his Creative Evolution, in which he argued that heredity and evolution were driven by an ‘élan vital’ or ‘vital impetus’ peculiar to the living.
Many scientists remained similarly unconvinced that the extraordinary dynamics of life and heredity could be accounted for by classical sciences such as thermodynamics, organic chemistry and physics.
2. The Organicists:
Another factor influencing the birth of quantum biology is more subtle and has to do with the philosophical movement of organicism that was popular with many of the leading scientists of the time. Organicism was a reaction to two opposing schools of thought in biology.
The first was mechanism, whose origins go at least as far back as the French Philosopher René Descartes, who maintained that all living organisms1 are essentially machines, differing in complexity but not in principle from those machines that had driven the Industrial Revolution.
The movement tended to be reductionist in the sense that it maintained that in biology, just as in all inorganic phenomena, the whole is no more than the sum of its parts. According to the mechanists, all life should ultimately be explainable in terms of the fundamental building blocks of matter and the forces that connect them, each obeying deterministic physical and chemical laws.
The opposing position to this is that of vitalism, which has deep roots in the religious and mythologies of the ancient world.
The organicists sought a middle ground.
They accepted that there was something mysterious about life but claimed that the mystery could in principle be explained by the laws of physics and chemistry—it is just that these had to be new laws, as yet undiscovered. One of the early proponents of organicism was Ludwig von Bertalanffy, who is generally accepted to have founded the interdisciplinary field called general systems theory, which has since been applied to everything from biology to cybernetics.
His work is considered to be among the forerunners of systems biology. In his 1928 book Kritische Theorie der Formbildung (Critical Theory of Morphogenesis) [23], he claimed that there was a need for new organizational principles to describe life.
His ideas influenced many other scientists, including the German physicist Pascual Jordan, who was one of the authors of the famous 1925 Dreimännerwerk (Three-man paper), together with Max Born and Werner Heisenberg.
This classic paper introduced the world to matrix mechanics, the mathematical framework on which quantum mechanics is built.
The following year, Jordan moved to Copenhagen to work with Niels Bohr.
In 1929, Bohr delivered a lecture to the Scandinavian Meeting of Natural Scientists entitled ‘The atomic theory and the fundamental principles underlying the description of nature’.
After mainly focusing on the successes of quantum mechanics in describing the nature of the atomic and subatomic world, he moved on to consider whether it might have something to say in biology:
Before I conclude, it would be natural at such a joint meeting of natural scientists to touch upon the question as to what light can be thrown upon the problems regarding living organisms by the latest developments of our knowledge of atomic phenomena which I have here described.
It was not clear what Bohr was hinting at with his remark about ‘the problems of living organisms’.
He was around this time still attempting to clarify his philosophical views, particularly on the measurement problem in quantum mechanics, as well as his ideas on ‘complementarity’, which we discuss further below. Indeed, in his 1929 lecture, he had emphasized in his typically vague way that the development of the atomic theory has… first of all given us a recognition of laws which cannot be included within the frame formed by our accustomed modes of perception; the lessons we have learned by the discovery of the quantum of action open up to us new prospects which may perhaps be of decisive importance, particularly in the discussion of the position of living organisms in our picture of the world.
But, despite the ambiguity of these words, Bohr was nevertheless a hugely charismatic and inspiring figure and his interest in the link between quantum mechanics and life encouraged Pascual Jordan to develop his own ideas further.
After returning to Germany, having taken up a post at the University of Rostock, Jordan maintained a regular correspondence with Bohr about the relationship between physics and biology over the next couple of years.
Their ideas culminated in what is arguably the first scientific paper on quantum biology. It was written by Jordan in 1932 and appeared in the German journal Die Naturwissenschaften; the article was entitled ‘Die Quantenmechanik und die Grundprobleme der Biologie und Psychologie’ (‘Quantum mechanics and the fundamental problems of biology and psychology’) [25].
Jordan incorporated the organicism approach into his thinking by claiming that life’s missing laws were the rules of chance and probability (the indeterminism) of the quantum world that were somehow scaled up inside living organisms.
He called this his ‘amplifier theory’ and based it on Bohr’s notion of the ‘irreversible act of amplification’ that is required in order to bring the fuzzy quantum reality into sharp focus by ‘observing’ it. Jordan believed that living organisms were uniquely able to carry out this amplification in a way that was conspicuously different from inanimate matter, such as a Geiger counter.
While this all seems somewhat vague—and it was—the important point is that Jordan was convinced he could extend quantum indeterminism from the subatomic world to macroscopic biology.
He even made a connection with free will by suggesting a link between quantum mechanics and psychology.
Jordan’s insistence that living organisms have a unique ability to amplify the quantum into the macroscopic world does have a lot of resonance with modern views of quantum biology.
However, he went much further and, in doing so, ultimately discredited the entire field by attempting to link his theories to Nazi philosophy in a mutual legitimization.
And unlike what was at least claimed by other German scientists, such as Heisenberg, Jordan’s political views were not merely those of a man capitulating to the insidiously hostile intellectual climate of 1930s Germany in order to ‘fit in’.
Jordan was genuinely sympathetic to fascism and Nazi ideology. Indeed, his biological speculations became increasingly politicized and aligned with Nazi ideology. He even claimed that the concept of a single dictatorial leader (Führer), or guide, was a central principle of life:
We know that there are in a bacterium, among the enormous number of molecules constituting this creature a very small number of special molecules endowed with dictatorial authority over the total organism; they form a Steuerungszentrum [steering centre] of the living cell.
Absorption of a light quantum anywhere outside of this Steuerungszentrum can kill the cell just as little as a great nation can be annihilated by the killing of a single soldier.
But absorption of a light quantum in the Steuerungszentrum of the cell can bring the entire organism to death and dissolution—similar to the way a successfully executed assault against a leading [führenden] statesman can set an entire nature into a profound process of dissolution. [25]
This attempt to import Nazi ideology into biology is both fascinating and chilling. Yet Jordan correctly pointed out that inanimate objects were governed by the average random motion of millions of particles, such that the motion of a single molecule has no influence whatsoever on the whole object.
This insight, as we will see, is usually credited to Erwin Schrödinger, who later claimed that life was different from inorganic chemistry because of its dependence on the dynamics of a small number of molecules.
Jordan similarly argued that the few molecules that control the dynamics of living cells within the ‘Steuerungszentrum’ have a dictatorial influence, such that quantum-level events that govern their motion, such as Heisenberg’s uncertainty principle, are amplified to influence the entire organism.
In August 1932, the same year that Jordan published his Naturwissenschaften paper, Niels Bohr delivered another key lecture, at the International Congress on Light Therapy in Copenhagen, Denmark.
Like Jordan, he was influenced by the organicists’ view that the mysterious ingredient of life was yet to be discovered; but rather than opting for quantum indeterminacy, Bohr claimed that the mystery ingredient was a quantum concept he had helped to conceive: complementarity.
Often referred to as wave–particle duality, this was for many of its founding fathers the central tenet of quantum mechanics. Indeed, for Bohr, the notion of complementarity went deeper than merely describing the dual nature of quantum entities, and he would later in life attempt to expand it into a broader philosophical notion.
But, in its simplest form, it can be applied, for example, to the nature of light, which can exhibit both wave-like and particle-like properties, but never both at the same time: the properties are complementary.
Bohr attempted to extend this notion into biology by arguing that there was an analogous complementarity between the functionality of life and our ability to study it.
On a fishing trip in the Baltic around 1932, Werner Heisenberg reports a conversation on Darwinian theory in which Bohr suggests the following: ‘On the one hand, it states that, through the process of heredity, nature tests [every new living form], rejecting the great majority and preserving a few suitable ones.
But there is the second assertion: that the new forms originate through purely accidental disturbances of the gene structure.
This claim is much more questionable …’ [27, p. 114]. Several decades and a world war later, in the early 1960s, Heisenberg was considering the same question when, at a meeting on the banks of Lake Starnberg in Germany and listening to a lecture about mutation and selection, he pondered whether, ‘something like intention were associated with Darwinian mutation.
We could ask whether the aim to be reached, the possibility to be realised, may not influence the course of events.
If we do that, we are almost back with quantum theory.
For the wave function represents a possibility and not an actual event.
In other words, the kind of accident that plays so important a role in Darwinian theory may be something very much subtler than we think, and this precisely because it agrees with the laws of quantum mechanics’ [27, pp. 242–243].
In 1931, another young German physicist, Max Delbrück, came to Copenhagen to work with, and be inspired by, Bohr.
Despite an early interest in nuclear physics, Delbrück became fascinated by biophysics and the emerging field of molecular biology in particular.
In 1935, he was one of three authors (the others being the Russian biologist Nikolay Timofeev-Ressovsky and the German biophysicist Karl Zimmer) of the landmark paper [28] that also became known as the Dreimännerwerk, in which they proposed that the idea of target theory, introduced by Friedrich Dessauer in Germany in the 1920s, could be used to deduce the size of a gene based on its susceptibility to ionizing radiation such as X-rays.
They assumed that a quantum of radiation would hit and affect a localized ‘target’ of just a few molecules within the cell. Their paper, ‘Über die Natur der Genmutation und der Genstruktur’ (‘On the Nature of gene mutation and gene structure’) would become the inspirational starting point for Erwin Schrödinger’s book, What Is Life? Delbrück went on to have a huge influence on molecular genetics.
He left Nazi Germany in 1937 and settled in America, where he eventually became a US citizen.
He won a Nobel Prize in 1969 for the discovery that bacteria develop resistance to viruses as a result of advantageous genetic mutations.
3. The Cambridge Theoretical Biology Club:
The interest in the physical basis of life was not limited to mainland Europe.
In the summer of 1932, an interdisciplinary group of scientists at the University of Cambridge set up the Theoretical Biology Club with the ambitious aim of solving ‘the great problem’ of whether life could be explained by the actions of atoms and molecules.
The group’s aim, like its counterparts in Germany and Austria, was to explore whether the ‘new physics’ (i.e. quantum mechanics) could provide novel laws in biology. It also hoped to merge reductionist biology with an organicist philosophy, though in this case inspired by the thinking of the great twentieth century philosopher Alfred North Whitehead.
Members of the group included some of the most influential scientists in early twentieth century biology, including biochemist Frederick Gowland Hopkins, who was awarded the Nobel Prize in Physiology or Medicine in 1929 (with Christiaan Eijkman for the discovery of vitamins), Joseph Woodger, who had translated Bertalanffy’s 1928 book into English, mathematician Dorothy Wrinch, who attempted to deduce protein structure using mathematical principles, developmental biologist Conrad Waddington, along with the great evolutionary biologist and geneticist J.B.S. Haldane. In 1934, Haldane wrote a paper entitled ‘Quantum mechanics as a basis for philosophy’ [29], which opens arguing that
Biologists have as yet taken but little cognizance of the revolution in human thought which has been inaugurated by physicists in the last five years.
It has been suggested that while the laws of physics are not violated in living organisms, life takes advantage of the uncertainty principle to make certain events more probable than they would otherwise have been.
He clarifies his position by arguing that, at the molecular level, life differs from inanimate matter in that it can be influenced on the macroscale by single events at the quantum level,
If bacteria are heated or poisoned with certain reagents, the number of survivors falls off exponentially.
This is taken to mean that the life of the cell depends on a single unstable molecule, whose change involves its death.
As the transformation of such a molecule involves the uncertainty principle, this principle plays a large part in the life of bacteria.
But higher organisms, even protozoa, behave as if their life depended on a number of similar molecules.
The uncertainty principle in this form plays a less important part in their lives.
They are protected from it by the laws of statistics, just as are large material particles consisting of many molecules.
By the closing years of the 1930s, a number of highly influential scientists on both sides of the Atlantic were examining the implications of the ‘new physics’ for biology, driven by a growing mechanistic picture of biology at the smallest scales, but under the umbrella of organicism. However, the Second World intervened to curtail any further progress.
Meanwhile, Pascual Jordan became increasingly politicized and evermore determined to link his ideas in quantum biology with Nazi ideology, with the conviction that, ‘after the victory, it could stand as a symbol and representation of the unbounded means of power of the new Reich’. In 1941, he published the book Die Physik und das Geheimnis des organischen Lebens (Physics and the Secret of Life), in which he continued to pose the question ‘Are the laws of atomic and quantum physics of essential importance for life?’
However, after Germany’s defeat, Jordan’s highly politicized ideas became anathema.
The other matchmakers of the proposed marriage between biology and fundamental physics were scattered to the four winds in the aftermath of the Second World War; and physics, shaken to its core by the atomic bomb, turned its attention to more traditional problems.
But the plans for a union were not entirely abandoned. One of the pioneers of quantum mechanics, Erwin Schrödinger, had fled Germany when the Nazis gained power.
He settled in Ireland, where in 1944 he published a book whose title posed the question ‘What is life? to which we now turn.
4. Order from order:
By the 1940s, it was known that heredity was governed by genes, but nobody yet knew what genes were made of. Schrödinger was impressed by the extraordinary high fidelity of genetic inheritance, which had been shown to be associated with mutation rates of less than 10–8 per gene per generation. He claimed that high fidelity of heredity could not be accounted for by the classical laws, because genes were too small.
Schrödinger’s argument starts from a consideration of the laws of classical physics and chemistry, such as those of thermodynamics or the gas laws.
He called these ‘order from disorder’ laws to reflect the fact that their orderliness is a product of underlying disorderly molecular dynamics.
He pointed out that their accuracy is limited by 1/√N, where N is the number of particles involved. So, a balloon filled with a trillion particles deviates from the strict behaviour of the gas laws by only one part in 1 million, thereby providing relatively accurate gas laws for such macroscopic systems.
However, a balloon filled with only 100 particles will deviate from orderly behaviour by one part in 10 (or 10% accuracy), and will thereby experience significant deviations from the gas laws.
For example, all the molecules in the balloon will sometimes, randomly, move towards its centre, causing the balloon to contract while at a constant temperature, thereby violating Boyle’s law.
This, he argued, created a problem in understanding the physical basis for the fidelity of heredity because genes were known to be too small to be subject to the order from disorder laws. Using target theory, he estimated the size of a gene as no bigger than a cube of sides 300 Å containing a maximum of about 1 million atoms, so the level of noise in heredity if based on the order from disorder principle should be about one in 1000, or 0.1%—clearly much higher than the observed mutation rates.
Schrödinger concluded that the accurate laws of heredity could not be founded on these order from disorder classical laws.
He argued that genetic information had to be encoded at the molecular level as ‘an unusually large molecule which has to be a masterpiece of highly differentiated order, safeguarded by the conjuring rod of quantum theory’.
Schrödinger called this principle on which he claimed life depended ‘order from order’, arguing that ‘incredibly small groups of atoms, much too small to display exact statistical laws, do play a dominating role in the very orderly and lawful events within a living organism’.
On the nature of genes, he claimed that genetic information must be encoded by a ‘more complicated organic molecule in which every atom, and every group of atoms, plays an individual role, not entirely equivalent to that of many others (as is the case in a periodic structure). We might quite properly call that an aperiodic crystal or solid.
Like Jordan’s amplifier principle, Schrödinger claimed that life was sensitive to the dynamics of small numbers of particles, and indeed, its structure and dynamics were encoded at the atomic level. He even suggested that ‘mutations are actually due to quantum jumps in the gene molecule, where here we must be clear that what Schrödinger meant by ‘quantum jumps’ is quantum tunnelling through a finite potential barrier, rather than the old notion of quantum jumps of electrons between energy levels.
Schrödinger’s book influenced both James Watson and Francis Crick, the co-discoverers of the DNA double helix, and was a factor in their decision to investigate the nature of genes. According to Watson, ‘this book very elegantly propounded the belief that genes were the key components of living cells and that, to understand what life is, we must know how genes act’. But the years following the publication of Schrödinger’s book saw the discovery of the DNA double helix and the meteoric rise of molecular biology, a discipline which developed largely without reference to quantum phenomena.
Gene cloning, genetic engineering, genome fingerprinting and genome sequencing were developed by biologists who, by and large, were content to ignore the mathematically challenging quantum world.
Physicists similarly dismissed the possibility that quantum effects could play a role in biology, particularly as demonstrating them in inorganic physical systems required an extraordinary level of control that could only be achieved by maintaining systems at temperatures close to absolute zero in a vacuum and shielded from environmental perturbations.
Quantum phenomena such as tunnelling or quantum interference effects depend on a system being well isolated from its surroundings. This was considered to be unsustainable for biologically relevant time scales within a hot, wet and complex system such as a living cell.
There were, however, occasional forays into the borderland between biology and quantum mechanics. When Watson and Crick published their structure of DNA they speculated that mutations could be caused by tautomerization of DNA bases from their common imino forms to the rare enol forms, which could produce incorrect base pairs during DNA replication.
The idea received a quantum twist from the Swedish physicist Per-Olov Löwdin, who proposed [32] that quantum tunnelling of protons could generate the tautomeric bases, thereby providing a physical mechanism for Schrödinger’s speculation that random point mutations might have a quantum origin. But few geneticists knew of or were influenced by Löwdin’s work.
Thus, the prevailing view by the 1960s—not only among biologists but among biophysicists and biochemists too—was broadly dismissive of the notion that quantum mechanics played any kind of special role in living systems.
An example of the attitude at the time can be found in the writing of Christopher Longuet-Higgins, a British theoretical chemist who made major contributions to molecular chemistry using mathematical modelling and analysis. In 1962, Longuet-Higgins wrote a paper entitled ‘Quantum mechanics and biology’, in which he was scathing of attempts to justify the importance of quantum mechanics in biology:
Nowadays, we smile at the concept of a ‘vital force’ governing the growth of living matter, but a few years ago it seemed that this force was coming back into biochemistry under the assumed name [of quantum biology] … One might envisage strange forces of a quantum mechanical nature…where atoms are deftly rearranged by some sort of tunneling effect.
But when a biochemist begins to use quantum-mechanical language in this nebulous way, we may justifiably suspect that he is talking nonsense. There was, I remember, some discussion a few years ago about the possible occurrence of long-range quantum-mechanical forces between enzymes and their substrates.
It was, however, perfectly right that such a hypothesis should be treated with reserve, not only because of the flimsiness of the experimental evidence but also because of the great difficulty or reconciling such an idea with the general theory of intermolecular forces.
Longuet-Higgins’s stance was very typical of the attitude towards quantum biology in the second half of the twentieth century, and, to a large extent, this scepticism was wholly justified.
Let us then summarize the role of the early quantum pioneers. The organicists, such as von Bertalanffy, were convinced that the deterministic classical laws of physics and chemistry were insufficient to account for the phenomena of life and that there was a missing ingredient yet to be discovered.
Quantum physicists, such as Bohr, Schrödinger and Jordan, took this as a cue and suggested that quantum physics was that missing ingredient. They seized on the notions of complementarity and the uncertainty principle to claim that measurement and quantum randomness may play a role in evolution, perhaps even providing some directional control to the evolutionary process.
However, this claim has largely been discredited and nearly all biologists remain wedded to the notion that there is no directionality in the mutational driver of evolution. What remained were vague ideas about a central role that some physicists such as Eugene Wigner ascribed to life, or rather to consciousness, as the magical ingredient necessary to solve the measurement problem. This idea has also been largely discredited.
On the other hand, both Jordan and Schrödinger identified a real point of contact between quantum and biological processes that is highly relevant to today’s work in quantum biology: macroscopic biological phenomena may be triggered by the dynamics of relatively small numbers of particles whose behaviour will be ruled or at least influenced by the non-trivial quantum phenomena such as uncertainty. Jordan wrote of a ‘very small number of special molecules endowed with dictatorial authority over the total organism; whereas Schrödinger insisted that ‘incredibly small groups of atoms … play a dominating role in the very orderly and lawful events within a living organism’.
Schrödinger went on to point out that this reliance on the dynamics of small numbers of particles separates biological systems with their order from order principle from macroscopic inanimate systems dominated by laws obeying the order from disorder principle. These ideas were picked up by some biologists, such as Haldane, who similarly insisted that ‘higher organisms, even protozoa, behave as if their life depended on a number of similar molecules’.
Although, reflecting the interests of their times, these quantum pioneers were particularly interested in the role of the uncertainty principle in life, their insights are transferable to the non-trivial quantum mechanical phenomena, such as coherence, tunnelling and entanglement, which are the focus of most modern quantum biology.
Also significant is Schrödinger’s claim that ‘The living organism seems to be a macroscopic system which in part of its behaviour approaches purely mechanical (as contrasted to thermodynamical) behaviour to which all systems tend, as the temperature approaches the absolute zero and the molecular disorder is removed’ [19, pp. 68–69]’.
In this, Schrödinger was essentially pinpointing the role of the randomizing influence of thermal motion, what we refer to today as ‘environmental decoherence’ [35], which is what separates the quantum from the classical world, an insight that is often traced back to the work of Dieter Zeh.
Schrödinger is essentially claiming that living systems somehow circumvent decoherence, an idea that resonates with modern work on the role that environmental noise may play in maintaining coherence in living cells.
However, by and large, most biologists continued to believe that life is adequately accounted for by all the familiar statistical laws of classical chemistry and physics. In 1993, a collection of eminent scientists from around the world (Steven Jay Gould, Lewis Woolpert, Stuart Kauffman and many others) gathered for a meeting at Trinity College in Dublin, Ireland, to celebrate the half-centenary of his famous 1943 lecture on which the book What is Life? [19] is based.
The book, What is Life: The Next Fifty Years [37], is a collection of essays written by the participants of the meeting. Yet, in most chapters, quantum mechanics is hardly, if at all, mentioned. Schrödinger’s bold proposal for a marriage between the disciplines appeared to have been forgotten.
During the 1960s and 1970s, there remained, however, a few physicists who entertained the possibility that quantum mechanics played a key role in biology. For example, the German-born British physicist Herbert Fröhlich proposed a theory in which quantum mechanical coherence, now known as Fröhlich coherence, plays an important role in biological systems.
A biological system that attains such a state of coherence is known as a Fröhlich condensate. He argued that biological organization was facilitated by coherent excited states at the molecular level, driven by the flow of energy provided by metabolic processes that generate molecular vibrations in terahertz range. While highly controversial, there is a current interest in testing this hypothesis experimentally using available sources of intense terahertz radiation.
5. Current thinking:
Despite Fröhlich’s work, most quantum physicists in the second half of the twentieth century became increasingly sceptical about the possibility of non-trivial quantum effects playing a significant role in biology, particularly from studies of open quantum systems and the role of decoherence in destroying the coherence necessary for non-trivial quantum effects.
From a theoretical perspective, a microscopic biological system, such as a biomolecular complex within a cell, must necessarily be treated as an open quantum system in the sense that it is never isolated from its environment.
Instead, it must be continuously supplied with energy from its surroundings to maintain its low entropy and out-of-equilibrium state, as well as being subject to the inevitable random thermal noise of its environment.
Therefore, it was expected that any delicate quantum effects, such as quantum superposition and coherence, will very rapidly dissipate (decohere), resulting in the suppression of any well-controlled quantum dynamics.
These considerations drew physicists and biologists (who considered the question at all) to conclude that quantum phenomena would be unlikely to play a significant role in biology.
Nevertheless, it is being increasingly recognized that, just as Jordan and Schrödinger argued, living systems may after all depend on the dynamics of small numbers of molecules that are extremely well localized (extending across just a few nanometres—the scale of biomolecules such as proteins) and can take place over very short time scales (often of the order of picoseconds).
This relative isolation in space, complexity and time could allow non-trivial, purely quantum mechanical processes to play an important role in living systems before decoherence induced by the surrounding environment can wash them away. There is now growing evidence that this is indeed the case.
The status of quantum biology changed dramatically in the last decades of the twentieth and early twenty-first centuries with sound experimental evidence for quantum coherence in photosynthesis and quantum tunnelling in enzyme action, together with strong theoretical arguments and some experimental evidence supporting the role of quantum entanglement in avian navigation and quantum tunnelling in olfaction.
Theoretical and experimental approaches have also explored the role of proton tunnelling in the generation of DNA base tautomers [14]. Figure 1 shows a timeline charting the major discoveries and publications in quantum biology.
Figure 1. Timeline of key landmarks in the development of quantum biology (QB) throughout the twentieth and early twenty-first centuries.
The boxes refer to the following sources: 1929 [24], 1932 [25], 1941 [20], 1944 [19], 1953 [42], 1963 [32], 1966 [43], 1974 [44], 1976 [45–48], 1989 [49], 2000 [50], 2007 [4]. FMO, Fenna–Matthews–Olson.
The past few years have seen a rapidly growing interest among a still small but expanding group of theoretical quantum physicists and chemists, experimental biochemists and spectroscopists who are carrying out serious theoretical and experimental studies of quantum effects in biology.
In fact, the situation is probably even more interesting than this. Work in quantum information theory has shown that environmental (thermal) noise in stationary non-equilibrium systems may actually support the existence of quantum coherence, allowing, as Schrödinger predicted, the dynamics of living systems to approach those of ‘purely mechanical (as contrasted to thermodynamical) behaviour to which all systems tend, as the temperature approaches the absolute zero and the molecular disorder is removed’.
Recent work has demonstrated that the retention of quantum dynamics in biological systems is intricately connected with environmental fluctuations taking place at biologically relevant length and time scales.
This recent research adds an extra layer to the insight of Jordan and Schrödinger that phenomena involving small numbers of particles subject to biological amplification (such as the hereditary material) were prime candidates for quantum biology.
The new research expands the role of quantum biology to more complex systems in which quantum dynamics might be enhanced, rather than washed away, by a finely tuned and constructive interplay between the quantum system and its surroundings.
Quantum biology has come a long way from the insights of the quantum pioneers of the early twentieth century.
Phenomena such as quantum tunneling and quantum coherence are now widely accepted as being involved in vitally important processes for all living cells, such as energy transfer and enzyme action.
The debate has now shifted from the question of whether quantum coherence and tunneling are involved to the role that they play.
Other areas of quantum biology, such as olfaction, magnetoreception or mutation, remain more speculative, at least partly because the experimental systems are not as tractable to precise physical measurement.
Content Source: Royal Society Publishing
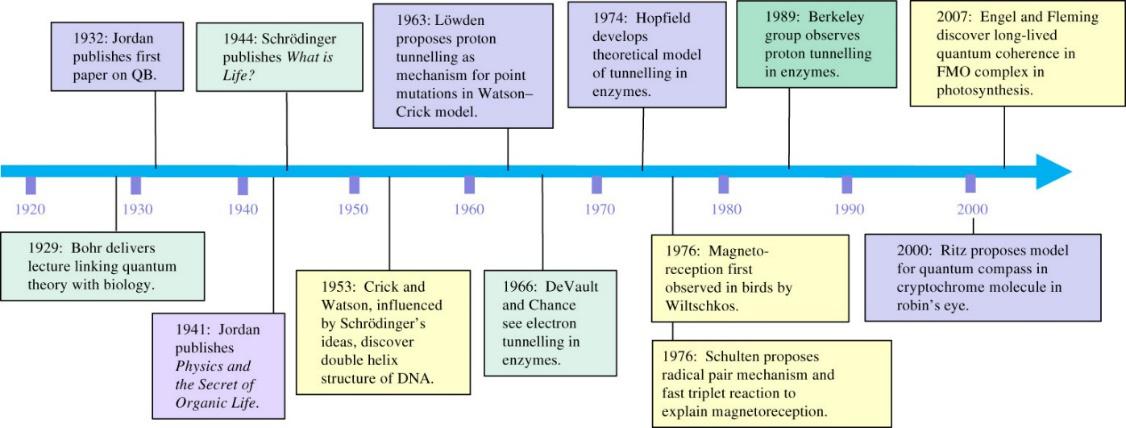
Email Us a Message 
Have a question about this article
Please send us a personal message below and we will serve you momentarily.
We appreciate you visiting the MMU Global Research Directory
For more blog posts, videos, articles, and to generate more knowledge, please feel free and…